Background
Analytical methods based on the chemistry of thiamine take advantage of its cationic charge via ion exchange for isolation and separation techniques; detection relying on its activity in the UV range; ability to be oxidized and detected via fluorescence, chemiluminescence, and electrochemiluminescence; and polarity differences between thiamine, its phosphate esters, and other species. While intact thiamine is highly water soluble, chemical or biochemical cleavage at the methylene bridge allows subsequent extraction of the less polar 4-methyl-5-(2-hydroxyethyl)thiazole fragment into organic solvents prior to GC analysis or quantification via scintillation counting if the thiazole fragment is radiolabeled. Biological-based methods take advantage of the fact that thiamine is an essential vitamin for growth for many microorganisms and as noted above, in its diphosphate form, serves as a co-factor for numerous enzymes. Modern methods rely on biologically derived molecules such as ribozymes and periplasmic binding proteins for specific recognition of thiamine.
Formation of thiochrome
While thiamine is visible in the UV range, its relatively common wavelength for absorbance (λ max = 242 nm) and low extinction coefficient do not lend to specific or sensitive analyses. Hence, most commonly, thiamine is converted to thiochrome (Figure 1) prior to measurement. This product of oxidation is highly fluorescent with excitation and emission wavelengths of 360 nm and 450 nm, respectively.
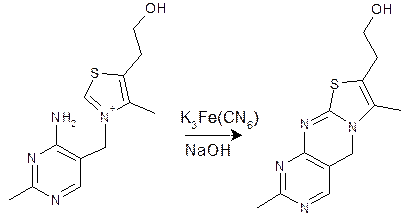
Oxidation commonly is carried out under alkaline conditions in the presence of potassium ferricyanide, although other oxidation conditions including cyanogen bromide and mercuric chloride have been used.
HPLC
Conversion to the fluorescent product thiochrome improves specificity towards thiamine detection versus UV detection. However, biological matrices often have constituents that undergo autofluorescence at the same wavelengths which require further sample preparation for quantification. High Performance Liquid Chromatography (HPLC) is often used to separate thiochrome from background constituents, as well as to allow speciation of thiochrome species formed from phosphorylated derivatives. Reverse phase chromatography is the most commonly employed mechanism, often using a polymeric poly(divinyl)benzene (PDVB) stationary phase rather than silica-based support, that can better withstand the high pH needed to maintain thiochrome fluorescence.
Clinical Assays
HPLC is commonly used to assess thiamine status in clinical samples, including whole blood, breast milk, and urine. However, biological activity assays are also carried out to assess deficiency. One commonly employed assay is the erythrocyte transketolase activity (ETKA) assay. In this method, the activity of transketolase, which catalyzes transfers of glycoaldehyde fragments from ketoses to aldoses, is assessed in the native sample, then reassessed after the addition of exogenous TDP. The glyceraldehyde-3-phosphate formed by transketolase catalysis is isomerized to dihydroxyacetone. The latter is then reduced to glycerol-3-phosphate with the concomittant oxidation of NADH to NAD+ (Figure 2). The rate of depletion of NADH is monitored at 340 nm.
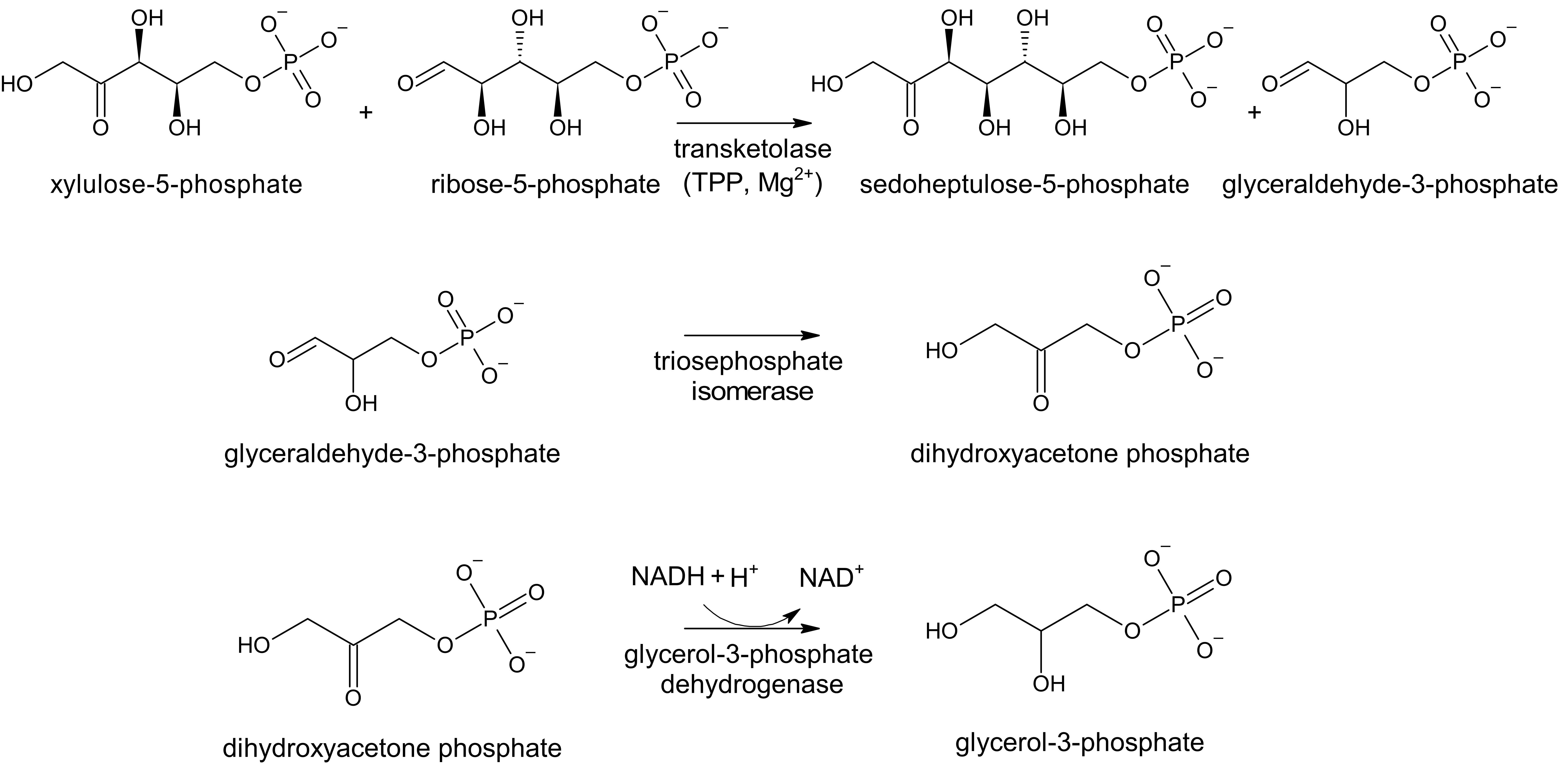
The baseline level of transketolase activity is compared to that after the addition of exogenous TDP, the difference in which is known as the thiamine pyrophosphate effect (TPPE). If there is sufficient TDP in the original sample, only a small increase in enzyme activity (0%-15%) is observed. However, if the original sample is TDP deficient, a larger increase in enzyme activity is observed (greater than 20%).
Microbiological Assays
Since many microorganisms require thiamine to sustain life, assays have been developed to monitor their growth in a quantitative manner with respect to thiamine concentration. Bacteria including Escherichia coli and Staphylococcus aureus, yeasts and fungi including Saccharomyces cerevisiae and Phycomyces blakesleeanus, and algae including Monochrysis lutheri have been used. The benefit to these assays is the low levels of thiamine required for growth, allowing for a low limit of detection. Unfortunately, many of these organisms not only respond to intact thiamine, but also constitutive fragments (thiazole and pyrimidine moieties) and can take multiple days to yield a result.
Bioanalytical Assays
Since thiamine is essential for the health of all organisms, analysts can take advantage of various biomolecules which specifically recognize and act on or sequester thiamine. For example, various enzymes use TDP as a cofactor, such as transketolase described above. Rather than monitor the level of endogenous activity as is done in clinical assays, one can instead use a purified form of the enzyme to monitor thiamine content. Other entities include periplasmic binding proteins expressed in bacteria and thiamine binding proteins found in various seeds. These molecules can serve much in the same manner as an antibody in an Enzyme-Linked Immunosorbent Assay (ELISA) to provide specific recognition. In fact, such entities are needed as antibodies against thiamine are challenging, if not impossible to develop. Shown on the right is a recent publication showing the use of a periplasmic binding protein expressed in E. coli for the detection of thiamine in a high-throughput format.
We demonstrated the use of this protein in a technique analogous to immunomagnetic separation (Figure 3). In this approach, the thiamine binding protein is conjugated to magnetic beads. The beads can readily diffuse throughout the sample and can selectively bind to thiamine, despite the presence of matrix interferences. The bound thiamine can be simultaneously released and converted to thiochrome for fluorescence detection using alkaline ferricyanide.
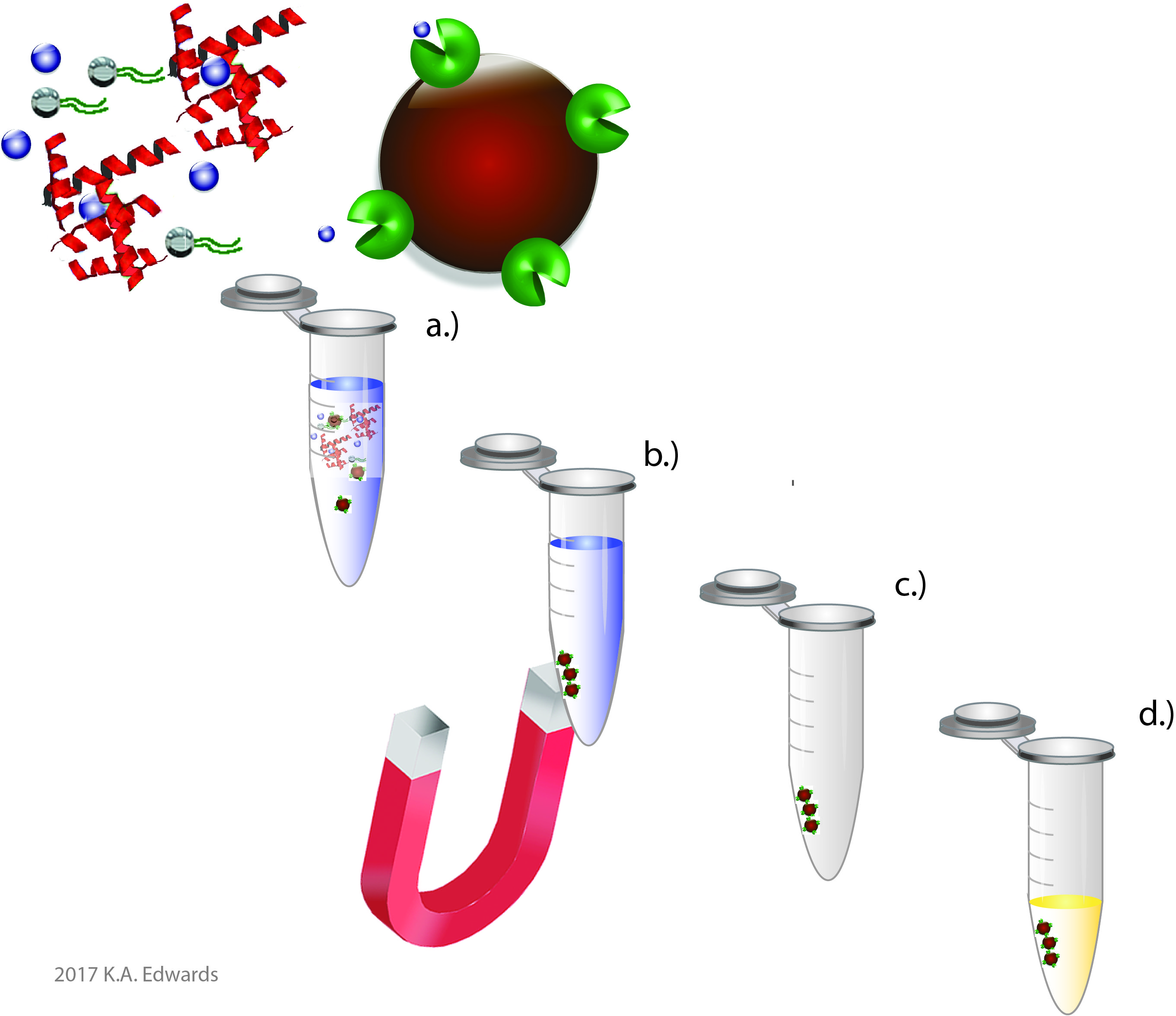